CFHT, Instruments,
Detectors,
IR, Redeye Manual: Chapter 2.
Below is a summary of some of the most important performance specifications
for both Redeye cameras.
Many of the questions users have about the cameras can be answered by simply
looking at this table. Note
that, even though the cameras are designed to support interchangeable parts,
in order to simplify summit
operations a particular set of optics and a cryostat was dedicated to each
array, hence users can find array
specifications based upon which camera they are using. All pertinent values
apply to the f/8 focus with a
1V bias voltage across the detectors (i.e., nominal conditions).

Table 2.1 - Many of the most critical performance levels of the Redeye
cameras are listed above. An asterisk indicates values that are only estimates
based on preliminary tests and will be updated with final values in V1.1 of
the manual.
Both cameras house NICMOS3 Hg:Cd:Te infrared array detectors. These devices
are manufactured by
Rockwell International Science Center. They are hybrid devices, consisting of
a photosensitive Hg:Cd:Te
layer that is bump bonded through Indium columns onto a low noise silicon
multiplexer. Each array has
256 x 256 pixels that are on 40
m centers,
yielding detectors that are 10.24 x 10.24 mm across. Unlike a
CCD, these detectors are literally composed of discrete photodiodes, with
charge switched out of the unit
cells and into one of 4 amplifiers located at the corners of the array. As a
result, NICMOS3 detectors do
not exhibit charge bleeding across rows or columns, as most CCDs suffer from,
making them easy to use
when bright sources are in the field. Each array is physically composed of
four 128 x 128 pixel subarrays
that are isolated from each other except for a common ground plane. This
permits a wide degree of
flexibility in tuning the performance of the device, as well as special
applications that demand, for
example, only single quadrant read-outs when data throughput is at a
premium.
Figure 2.1 shows bad pixel maps for the science grade arrays originally in
the cameras. A bad pixel in this
measurement is one that has a signal that differs from the average signal in
a flat field by more than a
factor of 2. For the narrow field science array, this corresponds to ~550 bad
pixels, of which ~90% are
dead (have 0 signal). Most of these pixels are single pixels scattered across
the entire chip, with a few
clumps. (Note that due to a failure of one quadrant of the narrow
field camera's array, a new device has been installed. As of this writing
engineering tests are underway in the lab and performance characteristics
will be determined on the telescope in the immediate future. As a result, all
references to the narrow field camera's detector performance in this
manual should be
disregarded until these data can be updated.) For the wide field camera's
array, there are ~150 bad pixels, with essentially all of these pixels
dead.

Figure 2.1 - Bad pixel maps of the science arrays are shown. To the left is
that housed in the wide field camera, to the right is the array in the
narrow field camera.
Figure 2.2 shows flat fields made with both science arrays. Note how these
devices exhibit "peaks" and
"valleys" of responsivity across there surface, presumably due to variations
in the thickness of the
Hg:Cd:Te layer after polishing. The wide field array has a
1
dispersion from the mean of ~8%, while the
narrow field array has a 1
dispersion from
the mean of ~10%. These variations are a function of array
bias, which is normally left at 1.0 V in order to make the well depth
~400,000 e-. Though this bias voltage
increases the read noise slightly, most Redeye applications will be broad
band imaging and will therefore
be background limited in a few seconds of exposure time with the read noise
induced by a 1.0 V bias.

Figure 2.2 - Flat fields for the wide field (left) and narrow field (right)
science arrays are shown.
It is important to realize that the Redeye detectors are sampled in a very
different manner than that used
with CCDs. The array is first reset to clear all charge from the wells and
then allowed to "settle" for a couple
of seconds. It is then read-out twice. The first read-out is discarded but
the second read-out is stored in
memory. Next, the shutter is opened, thereby starting an integration on the
sky. At the end of the
integration, the shutter is closed and all four quadrants are read-out in
parallel twice again. As in the
beginning of the exposure, the first read-out is discarded but the second one
is retained, then subtracted
from the read-out stored at the beginning of the exposure, a FITS header is
attached to the image, and the
file is stored on disk. As a result, there is no DC or bias offset left in
the image stored on disk, hence a 0
ADU value truly corresponds to 0 flux and dark current in the image. Implied
by this description of the
read-out process is the fact that NICMOS3 arrays have non-destructive
read-out architectures. While it is
theoretically possible to reduce the effective read noise in images through
repeated samples by a factor of
1/*n (until 1/f noise dominates), this introduces other deleterious effects,
described below.
NICMOS3 arrays have a number of peculiar characteristics that users should be
aware of when making
observations. These features stem from design flaws in the arrays and are
therefore found in all such
detectors. A detailed physical explanation for these problems with NICMOS3
arrays is not available, even
from the manufacturer, Rockwell. Hence our strategy has been to search the
operational parameter space
for the Redeye arrays and find read-out techniques that minimize the effects
of these device flaws. While
we are making every effort to reduce the impact of these problems through
sophisticated controller
algorithms, their effect is never eliminated completely in Redeye images.
Each of these features are
described in detail below.
At a low level, NICMOS3 arrays retain charge in pixels even after the devices have been reset. This
problem probably stems from charge trapping sites in the array's lattice structure that are not flushed by
reset pulses. The net effect of this phenomenon is that arrays retain a "memory" of past exposures. For
example, if a bright star is imaged and then several dark frames are recorded, faint residual images of the
star will be seen in the dark frames. Residual images typically retain only a ~1% percent of the flux level
in the original image, hence if a star saturates the array, the residual image would only have ~1% of the
full well charge in it. Residual images can be reduced through repeated reads and resets and they have a
bimodal decay nature. More specifically, the first residual image only has ~1% full well of charge, but
then the next read-out shows a reduction to ~0.3% full well, and subsequent images show a slow decay of
this remaining charge over dozens of frames. Figure 2.3 illustrates the fast and slow decay components in
a typical residual image. It was made by first flooding the array with light,
then recording a series of 30
second dark frames. In spectroscopic (i.e., low background) applications this
problem can be severe, since
residual images can last for hours at an extremely low level, regardless of
how many resets are issued.
Since Redeye is not used with filters that are narrower than ~1%, and is most
often used in broad band
high background situations, residual images are usually overwhelmed by
background flux and are
therefore not a major problem. Still, in an effort to minimize the problem,
the Gen III controller is
configured to send the NICMOS3 array in each camera a steady stream of reset
pulses when the camera is
idle. This stream of reset pulses also helps prevent the camera from
saturating when thermal (> 2.0
m)
filters are used, allowing the array to integrate on the back of the warm
external shutter. Furthermore,
observers are encouraged to make several reads before a set of darks is
acquired in order to flush as much
accumulated charge in the array as possible.

Figure 2.3 - A plot of residual charge as a function of frame number is
plotted. Note the steep drop in charge during the first few reads, then
the slow decay thereafter.
Observers may notice in some images a set of circular dark spots on the
arrays. At first glance, because
they are circular and have the appearance of being somewhat fuzzy or
"defocused", one might assume they
are caused by cold dust in the optics. Actually, these spots are intrinsic to
the arrays and probably stem
from slight nonuniformities in the doping of the devices. They are removed
from images through normal
image processing techniques. Interestingly, Hodapp et al. (1992) noticed that these spots correspond to
regions where residual image problems are minimized. There was speculation early after this discovery
that they were caused by gaps in the epoxy used to bond the photosensitive layer to the underlying silicon
multiplexer, but recent tests on a device intentionally left only partially glued proved otherwise. Since they
occupy such a small fraction of the array surface area, trying to beat the residual image problem by
placing targets in them is not practical.
Finally, one might suspect that this residual image effect can lead to accumulated charge over the course
of a night of observations, effectively reducing the dynamic range of the device and progressively
corrupting images at a low level. We have tested this possibility in the lab and found that the residual
charge does not accumulate beyond a fixed level in the arrays. In other words, if a set of images of a
galaxy are acquired with the camera in a simple staring mode, the flux is constant (within the various
noise sources) for the entire data set with a faint and constant residual image component superimposed on
all images.
A well known feature of NICMOS3 frames is the excess charge seen in the corners of images. This charge
comes from the output amplifiers in each quadrant during the read-out process and is believed to be due to
luminescence from the amplifiers. This flux source is only injected into images during read-outs, i.e.,
turning off the amplifiers during exposures has a negligible effect. While this problem is not a serious one
for typical high background Redeye applications, it is a serious noise source in low background
applications. For this reason multiple sampling schemes, though known to reduce read noise in frames
down to the 1/f noise limit, ultimately fail because the amplifiers must be repeatedly activated throughout
the sampling period, thereby greatly increasing the glow from the array corners and potentially
overwhelming object flux. Figure 2.4 shows the morphology of this glow in a dark frame. The high
frequency noise in this image is pick-up noise in the lab where this image was recorded.

Figure 2.4 - A short exposure showing glow from the output amplifiers
located on the corners of the array is shown.
If a NICMOS3 array is read-out immediately after resetting the device,
instead of the expected 0 ADU
signal level, a significant charge is seen across the entire array. This
charge decays very rapidly during a
"settling" process after the reset and is due to electrical coupling between
the reset signal and each pixel.
It is crucial that the array be allowed to settle after a reset and before
the first stored read-out in a double
correlated sample so this charge does not interfere with images. Users of
Redeye do not have to be
concerned with this facet of NICMOS3 arrays since the Gen III controller
pauses a couple of seconds after
sending a reset pulse to the array. It is only for time-critical
applications, where the array must be read-out
as rapidly as possible, that the reset anomaly becomes a significant
contributor to noise in images. And
ultimately determines how fast images can be recorded.
Unlike most silicon detectors, NICMOS3 arrays do not have strictly linear
dark currents as a function of
integration time. For short exposures the dark current is fairly constant
until 10 seconds of integration
time. From that point on dark current adds quasi-linearly over time. Also
note that when the camera has
been blanked off at the filter wheel, there are still extremely low level
light leaks that add to the signal
detected during dark exposures.
Since dark current does not add linearly with time, it is imperative that
observers not take short dark
frames and attempt to scale them by some simple factor before using them to
process frames. For example,
if a 10 second dark is multiplied by 10 before being subtracted from a 100
second object frame, the results
will be erroneous for at least two reasons. First, the dark current will not
be removed since the current is
not a linear function of exposure time. Second, as explained above, glow from
the amplifiers is a one-shot
injection of charge during each read and this charge would be over
compensated for if a scaled dark
current frame was subtracted during processing.
Figure 2.6 shows a number of aspects of the Redeye reimaging optics. First,
each camera system is shown
in perspective with the field lens in front and array in back. Rays from
three points in the field
corresponding to the on-axis, corner pixel, and mid-way point in the field
are shown. These rays are
drawn from the front of the field lens back to the detector. Also included in
Figure 2.6 is a large grey
square, representing the 2562 NICMOS3 array, with three smaller white squares
extending to the left.
These smaller squares represent, to scale, the 40
m pixels of the detectors. Spot diagrams for the
three
representative points in the field of view are shown in these pixels. Each
polychromatic simulation
includes flux from the blue, central, and red ends of the H band. In the case
of the wide field optics, a
slight (~1%) amount of flux falls outside of the 40
m bounds of a corner pixel while the narrow field
optics contain all of the reimaged flux in a single pixel. Corresponding
encircled energy diagrams are
shown in Figures 2.7 and 2.8. When telescope aberrations, tracking errors,
and seeing are convolved into
the image, it is clear that the Redeye optics should not be a limitation in
image quality, at least with the
current generation of 256 x 256 pixel arrays. When future arrays based on
smaller pixels and active
telescope optics are used with Redeye it may be possible to resolve a slight
amount of structure in the
Point Spread Functions (PSFs) created by the camera optics.

Figure 2.6 - Depicted above is a layout of the Redeye Narrow field optics,
while below is the wide field optical layout. Representative polychromatic
spots are shown for each model.

Figure 2.7 - An H-band encircled energy diagram for the narrow field optics
is shown. Point sources on-axis, 50% from corner pixel, and at corner
pixel are used.

Figure 2.8 - Same as Figure 2.7 except the wide field optical performance
is shown.
Though the lenses used in the Redeye optics are all made of high transmission
materials, they were still
coated in order to minimize internal reflections and secondary images or
ghosts when bright objects are in
the field of view. As part of the AR coating test procedure, LiF and BaF2
windows were coated and
scanned with a lab spectrometer to check their optical performance. A piece
of bare LiF was also scanned
as a control sample. Figure 2.9 shows the results of these tests. Note that
only one side of each window
was coated. From these scans it is clear that the coatings work fairly well
across the 1-2.5
m range (the
lab spectrometer did not work below ~1.3
m), with a
predicted roll-off at ~2.5
m. The
reflection per
surface, based upon these tests, is ~1%, averaged across the 1-2.5
m range. It should be noted that the
CaF2 Redeye windows were coated with the same material, meaning the
theoretical throughput of the 12
surfaces in each camera's optics is 0.9912 or 89%. Tests of these coatings
with astronomical images
indicate that they give exceptional rejection of internal reflections, with
> 10 mag rejection measured at
J (no ghosts were seen in any test images). Comparable performance is also
given at H and K, though the
demand for ghost rejection is in general reduced at these longer wavelengths
because of increased sky
brightness, which in turns leads to shorter exposures and lower contrast
between objects and the sky in
images.

Figure 2.9 - FTIR lab scans made of test LiF and BaF2 windows with the
same AR...
The performance of the Redeye optics was first measured through test masks
cut with LAMA consisting of
a grid of 20
m holes placed precisely in the
reimaging plane of the optics. Images made of these test
masks matched extremely well predictions based on computer models of the
narrow and wide field optics.
From there, imaging performance was evaluated via direct imaging through JHK
filters at the f/8
focus of CFHT, using globular clusters to provide a large number of point
sources across the field of view.
Figure 2.10 is one such image made of NGC 6553 through the wide field optics
in August 1992. This
particular image is actually the median of several dithered J-band images,
each 30 sec long. Table 2.2 lists
measured FWHM values from similar images made of globular clusters through
the narrow and wide field
optics. To generate these results, the CFHT program IQE was used to measure
the FWHM values of stellar
images in the globular cluster data across the entire field of view. The
systematic improvement towards
longer wavelengths is probably due to slightly improved seeing that
inherently comes from longer
wavelengths. The fact that the wide field optics yielded a PSF around 1" is
not surprising, since
digitization of the focal plane with the large (0.5") pixels created by this
mode will typically spread light
over a couple of pixels, no matter how good the seeing is. Consequently, the
~0.6" FWHM found with the
narrow field optics and ~1.0" FWHM with the wide field optics is
representative of what observers will
probably find during a typical night on Mauna Kea. On nights of superb
seeing, PSFs may drop by as
much as ~0.2" below these values, particularly at K.
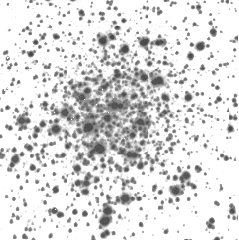
Figure 2.10 - An image of NGC 6553 using a J filter and the wide field
optics is shown. This and many other frames of globular clusters were
used in the image quality evaluation of the Redeye optics.

Table 2.2 - Image quality results with the narrow field and wide field
optics are listed.
Figure 2.11 shows, in more detail, the results of the image quality analysis
completed before the cameras
were commissioned. Each plot shows FWHM evaluations at J, H, and K across the
entire fields of view of
the narrow and wide field cameras. Based upon these data we concluded that
there is no significant
variation of image quality across the field of view for either the narrow or
wide field optics.

The plate scale for both modes was measured using binary stars at various
position angles. The wide field
optics were found to have a plate scale of 0.50" and the narrow field optics
have a scale of 0.20", as
designed. The change in plate scale as a function of wavelength is < 1
pixel across the entire field for
both modes, hence multicolor photometry of extended sources or globular
clusters should be
straightforward with the cameras.
Tables 2.3 and 2.4 list background and system throughput measurements made
with the science array in
August 1992. In each column a note regarding a "stop" is used. This refers to
a special occulting cold stop
that, when placed in the pupil image of the reimaging optics, blocks thermal
flux from the f/8
baffles and hole in the primary mirror from reaching the detector. Overall,
based upon these
measurements, we are getting very similar sensitivity levels as those found
by the other NICMOS3
cameras used on Mauna Kea. It is important to note that the use of the
occulting stops has no significant
impact on camera throughput and their only effect is beneficial in the form
of a reduced background in
images beyond ~2.0
m.

Table 2.3 - Relative sky background measurements are listed for 2 NICMOS3
cameras used on Mauna Kea. The term "stop" refers to a special occulting
stop that blocks thermal flux from the hole in the primary mirror
and f/8 baffles.

Table 2.4 - Relative system throughputs are listed for the same NICMOS3
cameras listed in Table 2.3. No significant reduction in throughput
was found with the occulting stop in place.
Hodapp, K-W., Rayner, J., and Irwin, E, "The University of Hawaii
NICMOS-3 Near-Infrared Camera," Pub. Astro. Soc.
Pacific, 104 (441).